Whilst it may not have the snappiest name, the event GW150914 is pretty significant in terms of our understanding of the Universe. This event, with a name that includes ‘GW’ as a prefix which is an abbreviation of ‘Gravitational Wave’ and the date of observation–15/09/14– marked humanity’s first direct detection of gravitational waves.
This was groundbreaking on two fronts; firstly it successfully confirmed a prediction made by Albert Einstein’s theory of general relativity almost a century before. A prediction that stated events occurring in the Universe do not just warp spacetime, but in certain cases, can actually send ripples through this cosmic fabric.
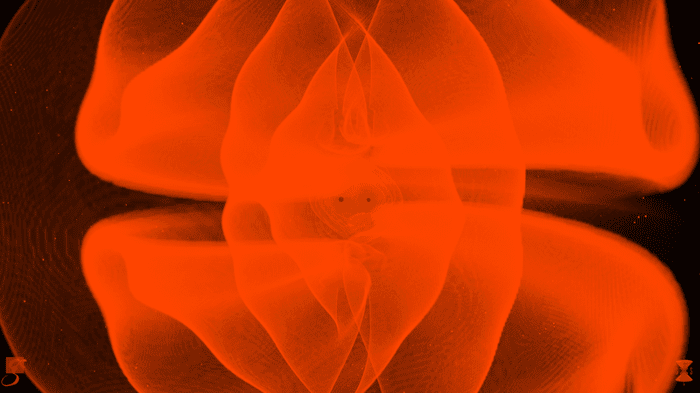
The second significant aspect of this observation was the fact that it represented an entirely new way to ‘see’ the Universe, its events and objects. This new method of investigating the cosmos has given rise to an entirely new form of astronomy; multimessenger astronomy. This combines ‘traditional’ observations of the Universe in the electromagnetic spectrum with the detection of gravitational waves, thus allowing us to observe objects that were previously invisible to us.
Thus, the discovery of gravitational waves truly opened up an entirely new window on the cosmos, but what are gravitational waves, what do they reveal about the objects that create them, and how do we detect such tiny tremblings in reality itself?
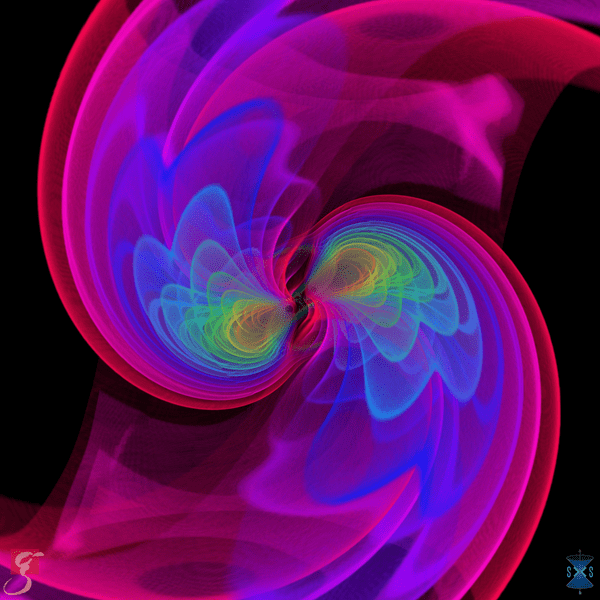
Gravitational Waves: The Basics
- Gravitational waves are ripples in the fabric of spacetime.
- These ripples travel from their source at the speed of light.
- The passage of gravitational waves squash and stretch space itself.
- Gravitational waves can be detected by measuring these infinitesimally small changes in the distance between objects.
- They are created when an object or an event that curves spacetime causes that curvature to change shape.
- Amongst the causes of gravitational waves are colliding black holes and neutron stars, supernovae, and stars that are undergoing gravitational collapse.
Theoretical Underpinnings
Imagine sitting at the side of a lake, quietly observing the tranquil surface of the water undisturbed by nature, the wind, or even by the slightest breeze. Suddenly a small child runs past hurling a pebble into the lake. The tranquillity is momentarily shattered. But, even as peace returns, you watch ripples spread from the centre of the lake diminishing as they reach the banks, often splitting or reflecting back when they encounter an obstacle.
The surface of the lake is a loose 2D analogy for the fabric of spacetime, the pebble represents an event like the collision of two black holes, and our position on Earth is equivalent to a blade of grass on the bank barely feeling the ripple which has diminished tremendously in its journey to us.
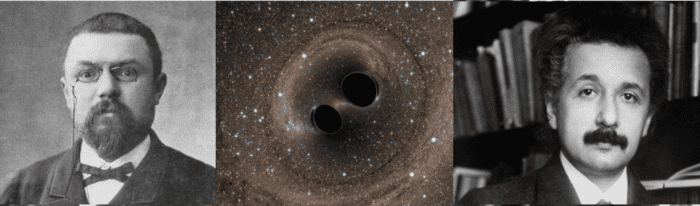
Gravitational waves were first predicted by Henri Poincare in 1905 as disturbances in the fabric of spacetime that propagate at the speed of light, but it would take another ten years for the concept to really be seized upon by physicists. This happened when Albert Einstein predicted the same phenomenon as part of his revolutionary 1916 geometric theory of gravity, better known as general relativity.
Whilst this theory is most well-known for suggesting that objects with mass would cause warping of spacetime, it also went a step further positing that an accelerating object should change this curvature and cause a ripple to echo through spacetime. Such disturbances in spacetime would not have been permissible in the Newtonian view of gravity which saw the fabric of space and time as separate entities upon which the events of the Universe simply play out.
But upon Einstein’s dynamic and changing stage of united spacetime, such ripples were permissible.
Gravitational waves arose from the possibility of finding a wave-like solution to the tensor equations at the heart of general relativity. Einstein believed that gravitational waves should be generated en masse by the interaction of massive bodies such as binary systems of super-dense neutron stars and merging black holes.
The truth is that such ripples in spacetime should be generated by any accelerating objects but Earth-bound accelerating objects cause perturbations that are far too small to detect. Hence why our investigations must turn to areas of space where nature provides us with objects that are far more massive.
As these ripples radiate outwards from their source in all directions and at the speed of light, they carry information about the event or object that created them. Not only this, but gravitational waves can tell us a great deal about the nature of spacetime itself.
Where do Gravitational Waves Come From?
There are a number of events that can launch gravitational waves powerful enough for us to detect with incredibly precise equipment here on Earth. These events are some of the most powerful and violent occurrences that the Universe has to offer. For instance, the strongest undulations in spacetime are probably caused by the collision of black holes.
Other collision events are associated with the production of strong gravitational waves; for example the merger between a black hole and a neutron star, or two neutron stars colliding with each other.
But, a cosmic body doesn’t always need a partner to make waves. Stellar collapse through supernova explosion–the process that leaves behind stellar remnants like black holes and neutron stars– also causes the production of gravitational waves.
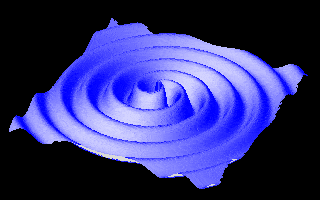
To understand how gravitational waves are produced, it is useful to look to pulsars–binary systems of two neutron stars that emit regular pulses of electromagnetic radiation in the radio region of the spectrum.
Einstein’s theory suggests that a system such as this should be losing energy by the emission of gravitational waves. This would mean that the system’s orbital period should be decreasing in a very predictable way.
The stars draw together as there is less energy in the system to resist their mutual gravitational attraction, and as a result, their orbit increases in speed, and thus the pulses of radio waves are emitted at shorter intervals. This would mean that the time it takes for the radio wave to be directly facing our line of sight would be reduced; something we can measure.
This is exactly what was observed in the Hulse-Taylor system (PSR B1913±16), discovered in 1974, which is comprised of two rapidly rotating neutron stars. This observation earned Russell A. Hulse and Joseph H. Taylor, Jr, both of Princeton University, the 1993 Nobel Prize in Physics. The reason given by the Nobel Committee was: “for the discovery of a new type of pulsar, a discovery that has opened up new possibilities for the study of gravitation.”
Though inarguably an impressive and important scientific achievement, this was still only indirect evidence of gravitational waves. Whilst the effect Einstein predicted of shortening of the pulsar’s spin was definitely present, this wasn’t an actual direct detection.
In fact, though not alive to witness this momentous achievement, Einstein had predicted that this would be the only way we could ever garner any hint of gravitational waves. The great physicist believed those spacetime ripples would be so faint that they would remain impossible to detect by any technological means imaginable at that time.
Fortunately, Einstein was wrong.
How do we Detect Gravitational Waves?
It should come as no surprise that actually detecting a gravitational wave requires a piece of equipment of tremendous sensitivity. Whilst the effect of gravitational waves–the squashing and stretching space itself–sounds like something that should pre-eminently visible, the degree by which this disturbance occurs is so tiny it is totally imperceptible.
Fortunately, there is a branch of physics that is pretty adept at deal with the tiny. To spot gravitational waves, researchers would use an effect called interference, something demonstrated in the most famous quantum physics experiment of all time; the double-slit experiment.
Physicists realised that a laser interferometer could be used to measure the tiny squashing and stretching of space as it would cause the arms of the equipment to shrink by a minute amount. This means when splitting a laser and sending it through the arms of an interferometer the squeezing of space caused by the passage of a gravitational wave would cause one laser to arrive slightly ahead of the other–meaning they are out of phase and causing destructive interference. Thus, this difference in arrival times causes interference that gives an indication that gravitational waves have rippled across one of the arms.
But, not just any laser interferometer would do. Physicists would need an interferometer so large that it constituents a legitimate feat in engineering. Enter the Laser Interferometer Gravitational-wave Observatory (LIGO).
The LIGO detector uses two laser emitters based at the Hanford and Livingstone observatories, separated by thousands of kilometres apart to form an incredibly sensitive interferometer. From these emitters, lasers are sent down the ‘arms’ of the interferometer which are actually 4km long vacuum chambers.
This results in a system that is so sensitive it can measure a deviation in spacetime that is as small as 1/10,000 the size of an atomic nucleus. To put this into an astronomical context; it is equivalent to spotting a star at a distance of 4.2 light-years and pinpointing its location to within the width of a human hair! This constitutes the smallest measurement ever practically attempted in any science experiment.
And in 2015, this painstaking operation paid off.
On 14th September 2015, the LIGO and Virgo collaboration spotted a gravitational wave signal emanating from the spiralling in and eventual merger of two black holes, one 29 times the mass of the Sun, the other 36 times our star’s mass. From changes in the signal received the scientists were also able to observe the resultant single black hole.
The signal, named GW150914, represented not just the first observation of gravitational waves, but also the first time humanity had ‘seen’ a binary stellar-mass black hole system, proving that such mergers could exist in the Universe’s current epoch.
Different Kinds of Gravitational Waves
Since the initial detection of gravitational waves, researchers have made a series of important and revelatory detections. These have allowed scientists to classify different types of gravitational waves and the objects that may produce them.
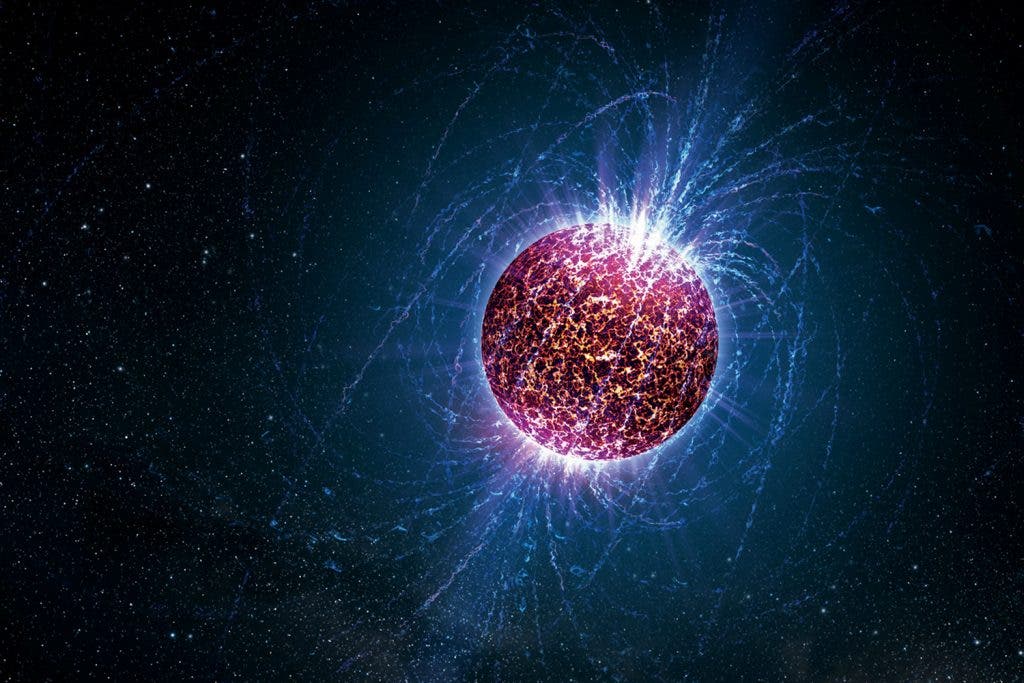
Continuous Gravitational Waves
A single spinning massive object like a neutron star is believed to cause a continuous gravitational wave signal as a result of imperfections in the spherical shape of this star. if the rate of spin remains constant, so too are the gravitational waves it emits–it is continuously the same frequency and amplitude much like a singer holding a single note. Researchers have created simulations of what an arriving continuous gravitational wave would sound like if the signal LIGO detected was converted into a sound.
The sound of a continuous gravitational wave of the kind produced by a neutron star can be heard below.
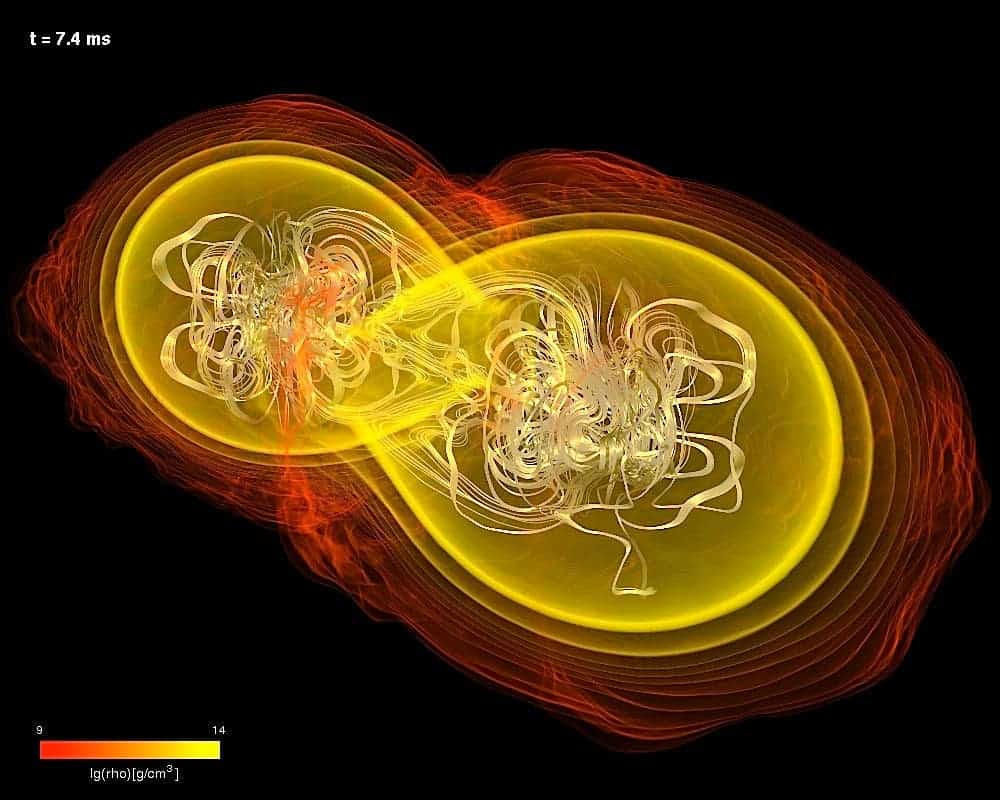
Compact Binary Inspiral Gravitational Waves
All of the signals detected by LIGO thus far fit into this category as gravitational waves created by pairs of massive orbiting objects like black holes or neutron stars.
The sources fit into three distinct sub-categories:
- Binary Black Hole (BBH)
- Binary Neutron Star (BNS)
- Neutron Star-Black Hole Binary (NSBH)
Each of these types of binary pairing creates its own unique pattern of gravitational waves but shares the same overall mechanism of wave-generation–inspiral generation. This process occurs over millions of years with gravitational waves carrying away energy from the system and causing the objects to spiral closer and closer until they meet. This also results in the objects moving more quickly and thus creating gravitational waves of increasing strength.
The ‘chirp’ of an eventual merger between neutron stars has been translated to sound waves and can be heard below.
(Albert-Einstein-Institut)
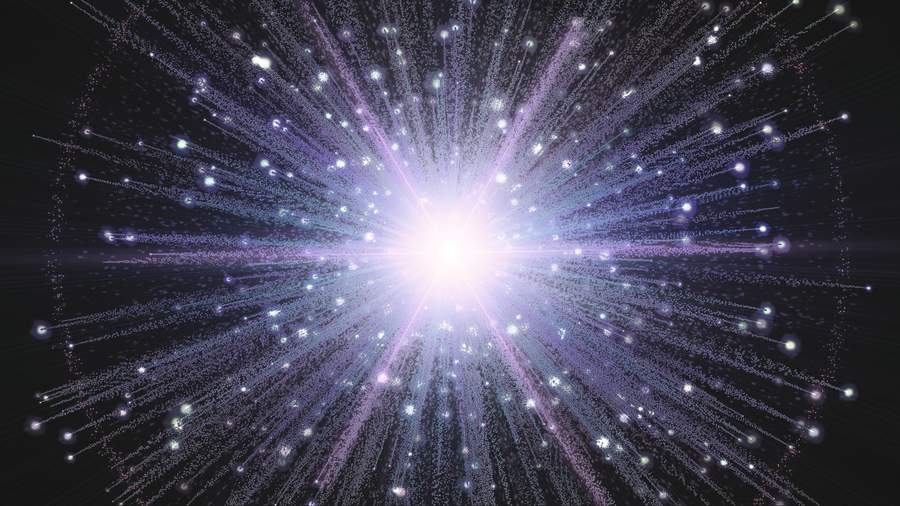
Stochastic Gravitational Waves
Small gravitational waves that even LIGO is unable to precisely pinpoint could be passing over Earth from all directions at all times. These are known as stochastic gravitational waves due to their random nature. At least part of this stochastic signal is likely to have originated in the Big Bang.
Should we eventually be able to detect this signal it would allow us to ‘see’ further back into the history of the Universe than any electromagnetic signal could, back to the epoch before photons could freely travel through space.
The simulated sound of this stochastic signal can be heard below.
It is extremely likely given the variety of objects and events in the Universe that other types of gravitational wave signals exist. This means that the quest to detect such signals is really an exploration of the unknown. Fortunately, our capacity to explore the cosmos has been boosted tremendously by our ability to detect gravitational waves.
A New Age of Astronomy
GW150914 conformed precisely to the predictions of general relativity, confirming Einstein’s most revolutionary theory almost exactly six decades after his death in 1955. That doesn’t mean that gravitational waves are done teaching us about the Universe. In fact, these ripples in spacetime have given us a whole new way to view the cosmos.
Before the discovery of gravitational waves, astronomers were restricted to a view of the Universe painted in electromagnetic radiation and therefore our observations have been confined to that particular spectrum.
Using the electromagnetic spectrum alone, astronomers have been able to discover astronomical bodies and even the cosmic microwave background (CMB) radiation, a ‘relic’ of one of the very first events in the early universe, the recombination epoch when electrons joined with protons thus allowing photons to begin travelling rather than endlessly scattering. Therefore, the CMB is a marker of the point the universe began to be transparent to light.
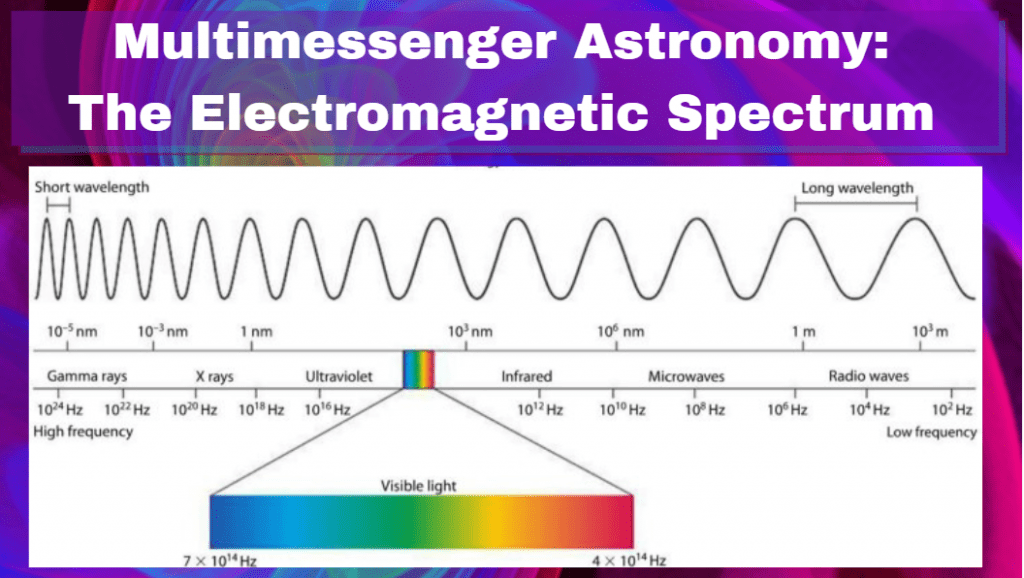
Yet despite the strides traditional astronomy has allowed us to make in our understanding of the cosmos, the use of electromagnetic radiation is severely limited. It does not allow us to directly ‘see’ black holes, from which light cannot escape. Nor does it allow us to see non-baryonic, non-luminous dark matter, the predominant form of matter in galaxies–accounting for around 85% of the universe’s total mass. As the term ‘non-luminous’ suggests dark matter does not interact with the electromagnetic spectrum, it neither absorbs nor emits light. This means that observations in the electromagnetic spectrum alone will never allow us to see the majority of the matter in the universe.
Clearly, this is a problem. But one that can be avoided by using the gravitational wave spectrum as both black holes and dark matter do have considerable gravitational effects.
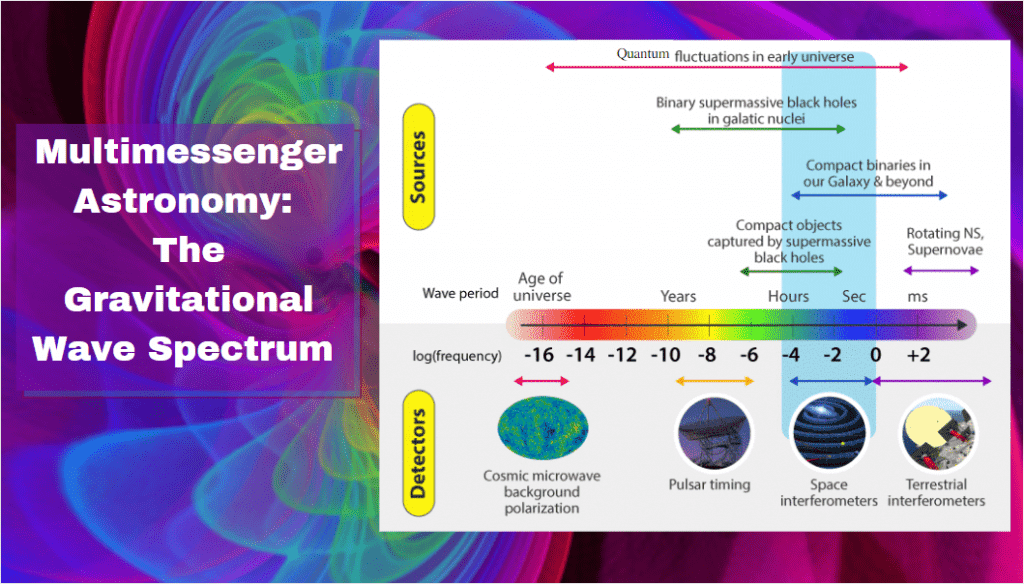
Gravitational waves also have another significant advantage over electromagnetic radiation.
This new form of astronomy measures the amplitude of the travelling wave, whilst electromagnetic wave astronomy measures the energy of the wave, which is proportional to the amplitude of the wave squared.
Therefore the brightness of an object in traditional astronomy is given by 1/distance² whilst ‘gravitational brightness’ falls off by just 1/distance. This means that the visibility of stars persists in gravitational waves for a much greater distance than the same factor persists in the electromagnetic spectrum.
Of course, none of this is to suggest that gravitational wave astronomy will ‘replace’ traditional electromagnetic spectrum astronomy. In fact the two are most powerful when they are unified in an exciting new discipline–multimessenger astronomy
Sources and Further Reading
Maggiore. M., Gravitational Waves: Theory and Experiments, Oxford University Press, [2019]
Maggiore. M., Gravitational Waves: Astrophysics and Cosmology, Oxford University Press, [2019]
Collins. H., Gravity’s Kiss: The Detection of Gravitational Waves, MIT Press, [2017]
Look Deeper, LIGO, [https://www.ligo.caltech.edu/page/look-deeper]