By the mid-20th Century, physicists had begun to understand the fundamental structure of matter to such a degree that a theory was needed to encapsulate the Universe’s particles, the interactions between them and the forces that govern those interactions. That theory was the Standard Model of Particle Physics or just the Standard Model for short.
First devised in the 1970s, the Standard Model would be used to predict a wide variety of phenomena, meet various experimental challenges, before being confirmed by the discovery of the Higgs Boson in 2012. Yet, as successful and fruitful a theory as the Standard Model is, it can’t explain everything. Gravity still evades confinement within the Standard Model, and physicists have caught tantalizing glimpses of physics beyond the theory’s limits.
Before these glimpses can be confirmed and a new chapter in physics is opened, let’s take a trip through the particle zoo and discover the wonders of the Standard Model.
The Matter Particles
The everyday matter that surrounds us is comprised of building blocks called elementary particles. Of these building blocks, there are two main families; fermions and bosons.
Of the fermions, the two mains classes are leptons and quarks. Within each of these groups are six particles that group into three pairings that physicists call generations.
The first generation of leptons and quarks are made up of the lightest and most stable particles. These are the particles responsible for forming the elements of the Universe we are most familiar with–the stars, planets, moons, and us. The second and third generations are made up of increasingly more massive and less stable particles. The greater in mass these particles are, the quicker they decay into their lighter cousins.
Starting with the quarks is an easy way to introduce some of the qualities and values associated with the particles in the Standard Model. One thing you will notice is the interesting naming convention for these qualities. They reflect things we commonly encounter in the everyday macroscopic world such as flavour, colour and spin, but really shouldn’t be confused with those things.
So, let’s make quarks the first stop in our walk through the particle zoo.
Quarks
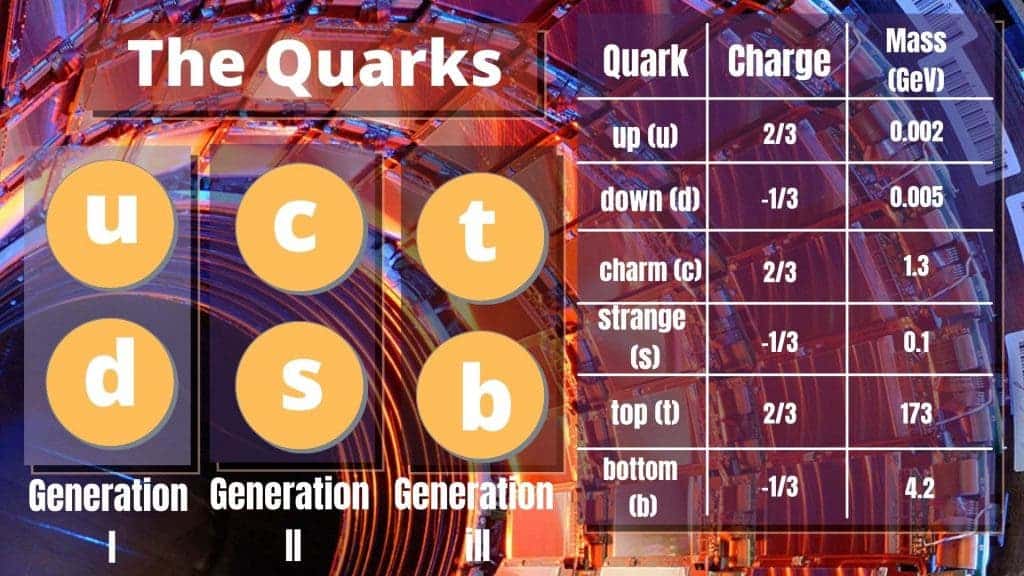
The six quarks that make this family of particles are known as up, down quarks, which make up the first generation of quarks, these second comprises of the more massive charm and strange quarks. And the third generation contains the most massive particles, known as the top and bottom quarks.
These are generally known as the ‘flavours’ of quarks, each of which has its own antiquark. Of course, I’ve primed you to realise that this name has nothing to do with how these quarks taste!
Of the four fundamental forces, quarks ‘feel’ electromagnetism, the strong and weak nuclear forces, and gravity, but the latter is too weak to have an effect on quarks’ tiny mass.
The strong nuclear force binds together quarks in nucleons, whilst the weak nuclear force can actually cause quarks to switch flavours something that we’ll look at further when we get to the force-carrying particles.
But these elementary particles don’t just come in flavours–they also come in ‘colours.’
It is this quality–again nothing to do with wavelengths of light, quarks are large enough to reflect light in such a way to have a conventional colour– that determines how quarks come together to form other, more massive, particles.
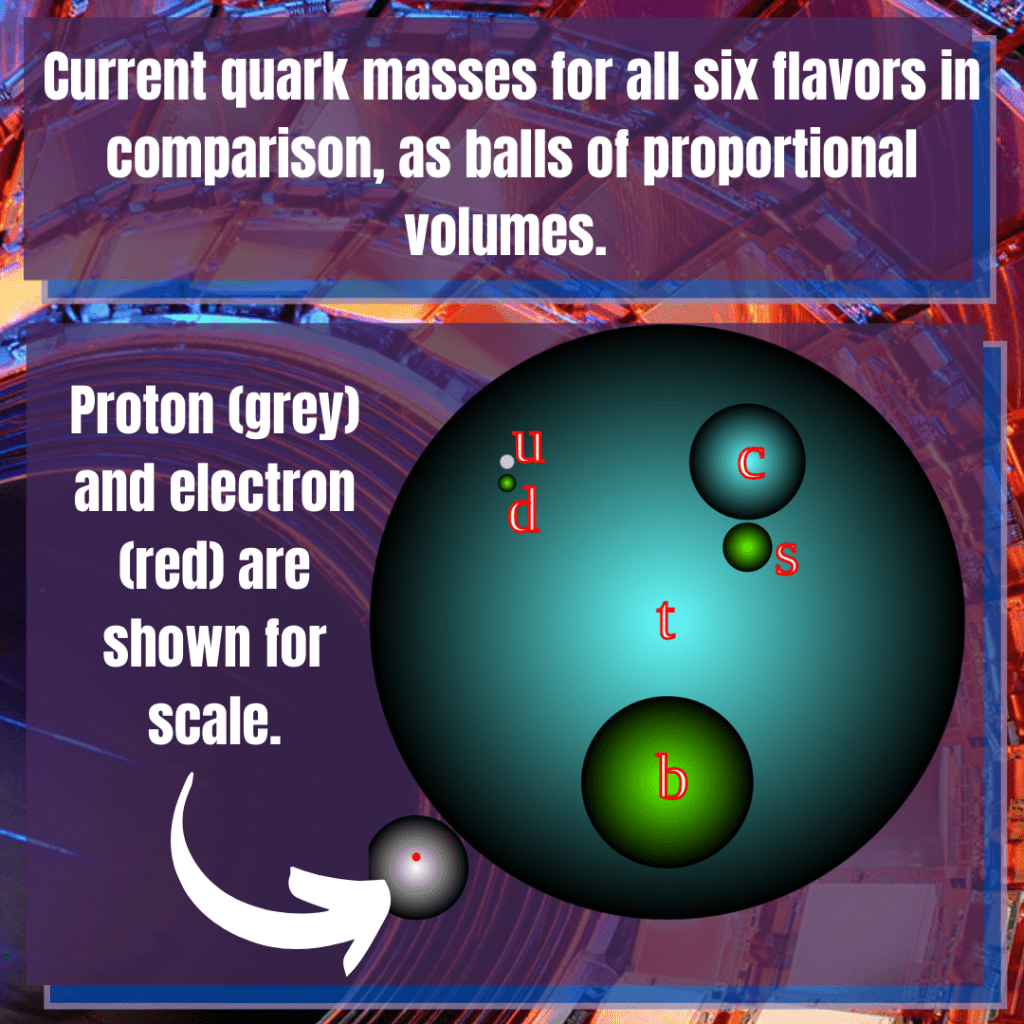
Quarks join up to make particles called baryons, the most common of which are the protons and neutrons that come together to form the elements and the matter we interact with on an everyday scale.
Protons are made up of one down quark and two up quarks, whilst neutrons are comprised of two down quarks and an up quark.
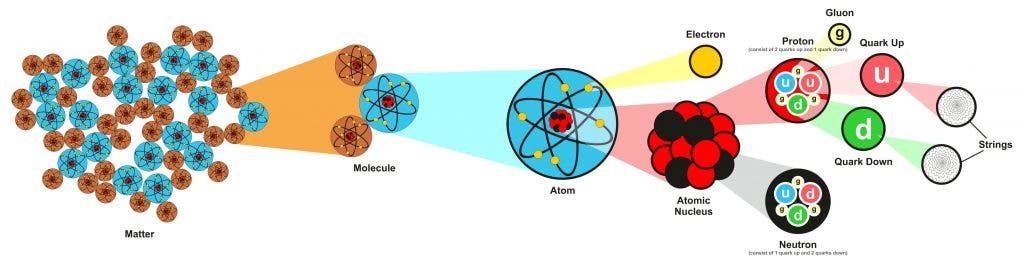
Considering these arrangements and the fact that each flavour of quark has its own charge it’s easy to see why the proton has a positive charge whilst the neutron is neutral. It should also be apparent that when the weak nuclear force causes an up quark to switch to a down quark it also charges the nucleon it is part of from a proton to a neutron.
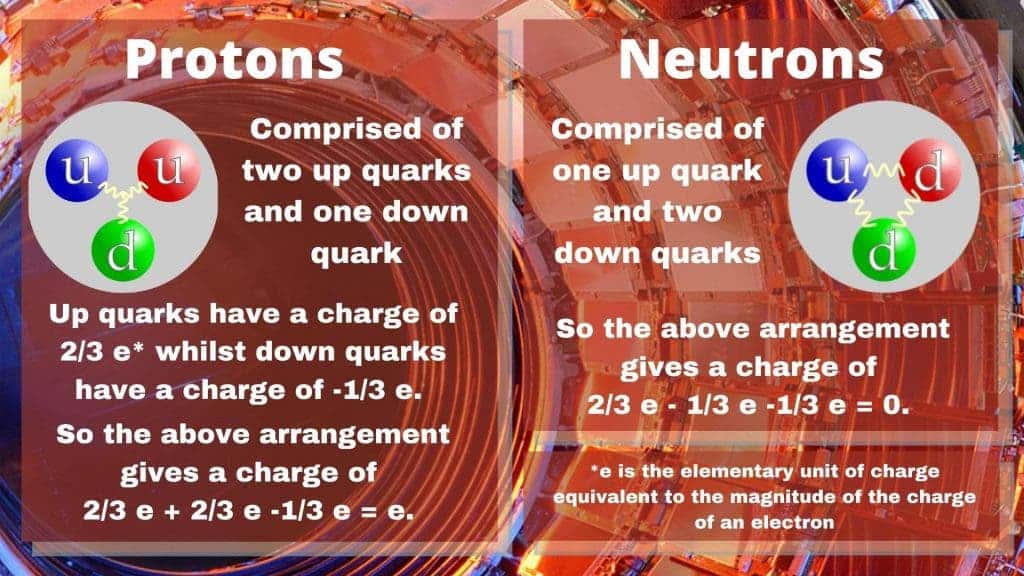
There are a multitude of other exotic arrangements of quarks like mesons which consist of a quark and its antiquark, and tetra and pentaquarks made up of three and five quarks respectively.
Considering how quarks come together to form particles is important because despite being fundamental particles, quarks are found wandering the particle zoo on their own. They are always found in conglomerations.
There is another important quality of fundamental particles that need to be considered–and yes, just as with ‘flavour’ and ‘colour’ it has a slightly misleading name.–these particles also have ‘spin.’
This shouldn’t be considered as representing a particle constantly revolving. It’s more a description of how a particle reacts when it interacts with a magnetic field.
Quark, like all fermions, are 1/2 spin particles and are described as having ‘up’ or ‘down’ spin. Unlike the spin of a macroscopic object, say a football after it is kicked, the spin of fundamental particles doesn’t change.
Leptons
Like quarks, leptons are particles with 1/2 spin. They also come in six flavours and across three generations. But, unlike quarks, leptons are freely found wandering the particle zoo alone. The most famous lepton is possibly also the most famous fundamental particle. The electron–a generation I particle possessing a charge of –e.
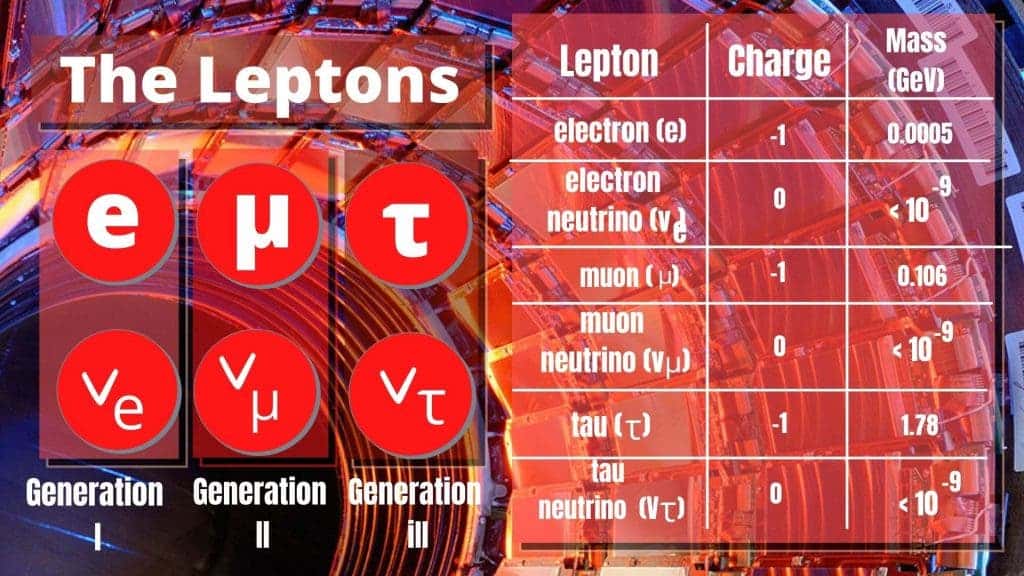
Leptons can also be sub-divided into two groups; charged, which includes electrons and electron like muons, and chargeless leptons like the neutrinos. The charged leptons also possess a more considerable mass than their uncharged cousins. The reason that uncharged leptons have such smaller masses is not explained by the Standard Model of Particle Physics and doing so requires an extension to the model.
The lack of charge and practical lack of mass of neutrinos has led them to be labelled ‘ghost particles’ and means that 100s of thousands of them can stream through every square inch of your body every second without the slightest interaction with the matter that composes you.
Just like with quarks, each particle has its own anti-particle, including perhaps the most famous example of such symmetry–the antiparticle of the electron, the positron. One possible quirk to this symmetry is the possibility is that neutrinos are their own antiparticles.
Like quarks, leptons interact with gravity and the electromagnetic force, but unlike quarks leptons don’t feel the strong nuclear force.
Leptons obey the Pauli exclusion principle. This means that no two particles can share the same quantum numbers. This is key to the range of chemical elements that exist within the Universe as it forces electrons to occupy increasingly energetic shells around an atomic nucleus. The number of valance electrons in an element’s outer shell determines the chemical properties that the element will have.
The Pauli exclusion principle can be overwhelmed. A neutron star is protected from becoming black holes by this phenomenon, but when it exceeds a certain mass it can no longer rely on this to protect against complete gravitational collapse.
The Force Carriers
There are four fundamental forces in the Universe that we are currently aware of–the strong force, the weak force, the electromagnetic force, and the gravitational force. All of these forces work over different distances with different strengths. For instance, gravity is the weakest of the forces–even though there is actually a force explicitly called the ‘weak force’– but works over a potentially infinite distance. Meanwhile, the electromagnetic force also works over a long-range but is much more powerful than the force of gravity.
The strong and weak nuclear forces work over much shorter ranges; dominating the forces for sub-atomic particles. As the name implies, the strong force is the strongest of all the four forces, whilst the weak force is the weakest barring gravity.
We are certain that three of the four fundamental forces–electromagnetism, the strong and weak forces–are communicated by carrier particles called bosons. Particles exchange these bosons to communicate these forces.
Unlike leptons and quarks–collectively known as Fermions–Bosons have full integer spin. This means that they are not forced to obey the Pauli exclusion principle.
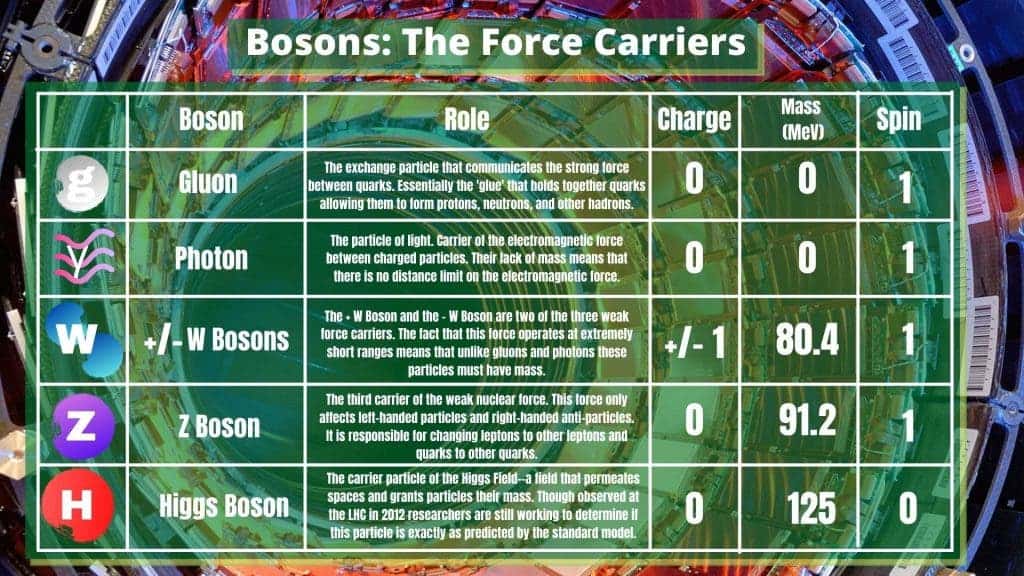
The electromagnetic force is carried by the most familiar of these particles–the photon. The strong force which ‘glues’ quarks together in protons and neutrons is communicated by gluons, and the weak force that influences particles to switch flavours is transmitted by W and Z bosons.
So what about gravity?
Put simply, the force that we are most familiar with and experience every moment of every day isn’t part of the Standard Model. Physicists think that this outsider force is also transmitted by a boson which they have given the provisional name the graviton. As of yet, however, there is no experimental sign of this hypothetical boson. Thus it can’t yet be seen in our particle zoo.
The exclusion of gravity isn’t a massive problem for particle physics, because the model deals with particles that are so small and the fact that gravity is so weak, the force doesn’t really have an effect on this sub-atomic world.
But what this omission does tell us, is that despite its importance and the fact that it has been experimentally verified to an impressive standard, and can now predict that outcome of a wide array of experiments, the Standard Model is by no means a complete description of the physical world.
That means we need extensions to this model to obtain that more accurate description. The problem is, no one can quite agree on what those extensions should look like.
Beyond the Standard Model
The force of gravity isn’t the only element of the Universe that physicists can’t squeeze into the Standard Model at the moment. Despite being a great description of sub-atomic particles, the theory can’t account for dark matter. As this mysterious form of matter that isn’t made up of baryons like protons and neutrons, accounts for around 85% of the mass in the known Universe, this isn’t an insignificant shortfall.
Likewise, the model can’t explain why matter dominates the Universe rather than antimatter. Processes that birth particles produce matter and antimatter in equal amounts. If the Universe had started with these balanced they would have likely met and annihilated each other before large scale structure had the oppotunity to form. That means there must be some reason beyond the Standard Model why the Universe initial favoured matter and allowed an imbalance.

Another potential issue with the Standard Model could result from the particle that was heralded as marking its completion: the Higgs Boson.
This particle is believed to emerge from the Higgs field and endow mass to most particles. But, the Standard Model isn’t the only theory that posits the existence of the Higgs Boson. The Higgs particle suggested by this theory is the simplest version. The particle that was measured by the CMS detector at the Large Hadron Collider (LHC) certainly conforms to the description given by the Standard Model, but it’s not a perfect fit.
That means that even as we create more Higgs Bosons at the LHC and continue learning more about the particle, the possibility of discovering that it conforms better to another theory remains.
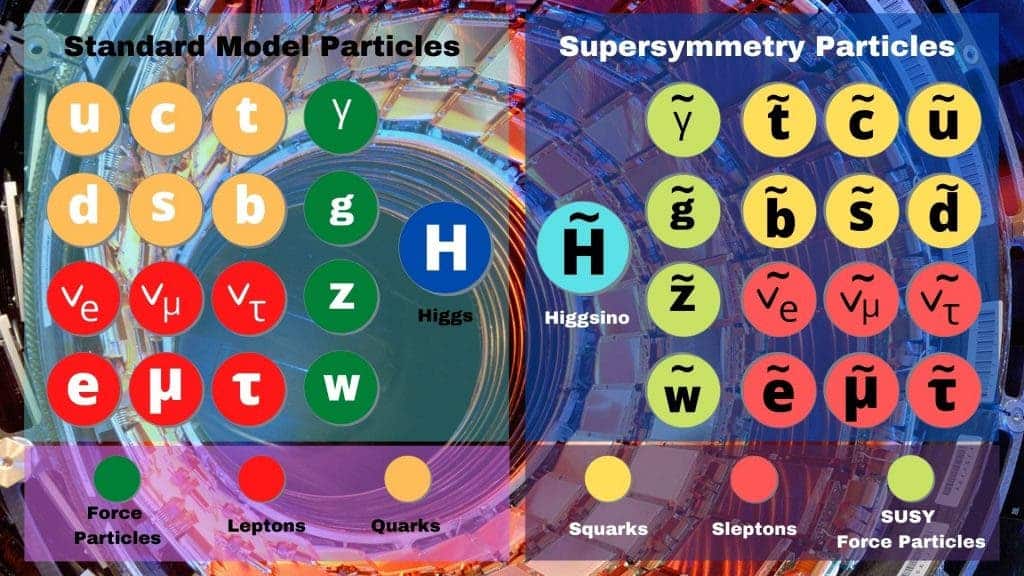
One of the most well-supported extensions to the Standard Model is Supersymmetry (SUSY). This hypothesises a connection between fermions and bosons and suggests that all particles have a superpartner –or sparticle–with the same mass, and quantum numbers but a spin that differs by 1/2.
That means that each 1/2 lepton is a partner ‘slepton’ with a full integer spin–or more simply a boson. So, for the electron, SUSY posits the slepton with the same mass, charge, but with a spin of 1 rather than 1/2 called the selectron. For quarks, there are squarks, and so on.
SUSY could provide a dark matter candidate as the lightest particle suggested by the extension to the Standard Model would, if it existed, be a dead-ringer for dark matter.
Unfortunately, despite some tantalising hints at physics beyond the Standard Model of Particle Physics, experiments have thus far failed to turn up anything substantial. For SUSY specifically, sparticles that should be created in collisions at the LHC have thus far not been detected.
At least until the completion of high-luminosity upgrades at the LHC provide more collisions and thus a greater chance of spotting exotic phenomena, the Standard Model will remain our best, albeit incomplete, description of the sub-atomic world.
Sources and Further Reading
Manton. N., Mee. N., The Physical World, Oxford University Press, [2017].
Martin. B. R., Shaw. G., Particle Physics, Wiley, [1999].