
It’s one of the first things we learn: plants utilize carbon dioxide (CO2) and water to make food through a process known as photosynthesis. While we’re typically taught that water is absorbed mostly through the ground, we often overlook the carbon assimilation part. This process, known as carbon fixation, involves assimilating carbon and converting it into organic, usable compounds.
Why is Carbon Fixation Significant?
Plants absorb water from the ground. So, what’s the big deal with absorbing CO2? Why is it a complex multi-step process? Well, because carbon isn’t just assimilated—it’s also transformed.
The ABCs of Carbon Fixation
Carbon Capture

Plants, including algae, primarily absorb carbon from the air. They take in CO2 from the atmosphere through their stomata — tiny kidney-shaped pores on the surface of their leaves. This CO2 uptake is what enables them to produce their version of food — glucose (a sugar).
Unlike water, the process of assimilation doesn’t end here. This is because atmospheric CO2 is useless. Plants can’t do anything with it in its current inorganic form. To utilize the collected CO2, they must first convert the molecular carbon into an organic form. Quite literally, plants need to fix CO2.
Fixing Carbon
At its core, carbon fixation is a chemical process, and like most chemical processes, it is catalyzed by an enzyme– biomolecules that affect the rate of a reaction. In the case of carbon fixation, the primary enzyme involved is Ribulose-1,5-bisphosphate carboxylase/oxygenase, or rubisco.
If the name sounds like an unnecessary mouthful, you can blame biochemical nomenclature rules. According to the International Union of Pure and Applied Chemistry (IUPAC) conventions, enzyme names originate from the substrate or the chemical reaction they facilitate. In this case, the enzyme acts on the molecule Ribulose-1,5-bisphosphate, or RuBP. As for the reaction, you may have guessed that carbon fixation involves the addition of either carbon dioxide or oxygen to RuBP.

Carboxylation: A Key Step in Sugar Production
Although rubisco can add either molecule — molecular oxygen or CO2 — the primary function of Rubisco in photosynthesis is carboxylation, leading to the fixation of carbon dioxide into organic molecules like carbohydrates. The enzyme facilitates the carboxylation of RuBP, which ultimately leads to the production of organic molecules such as glucose.
If that last bit got you confused a bit, think of it this way: Imagine RuBP as a building block with two holes on its surface. Carboxylation is like taking a single Lego brick (CO2) and snapping it securely into one of those holes on the RuBP block.
Essentially, when we talk about carboxylation — in the context of photosynthesis — we’re referring to when a CO2 molecule is attached to a RuBP molecule, in the presence of rubisco. This changes the shape and properties of the RuBP, allowing it to be used in the next steps of sugar production.
What Happens Next?
While all plants capture and use CO2, they don’t all process it in the same way. The fate of atmospheric CO2, once taken in through the stomata, classifies plants into three major types: C3, C4, and CAM.
C3 Pathway
This pathway is relatively straightforward. After CO2 is captured from the atmosphere, it combines with RuBP to form two molecules of 3-phosphoglycerate (3-PGA). This molecule is the first stable organic compound produced during carbon fixation. Because 3-PGA is a three-carbon compound, the pathway is named C (for carbon) 3 accordingly. The majority of plant species (around 85%), including crops, trees, and shrubs, utilize the C3 carbon fixation pathway during photosynthesis.
Common Not Perfect
In ideal conditions, most—if not all—plants opt for the C3 pathway yet, ideal conditions are rare. This is especially true in dry regions, where resources are rare and conditions harsh. Take, for instance, the dilemma of carbon capture. It relies on open stomata, yet this very action also paves the way for water loss—a precious commodity in such environments.
Remember how rubisco can pick up either molecular CO2 or oxygen? That’s not a good thing. When molecular oxygen levels increase, rubisco snatches up the surplus amount and instead of carboxylation–the first step of carbon fixation–takes up photorespiration instead.
In photorespiration, instead of processing CO2, plants end up releasing CO2 instead of using it. To conserve resources, plants in such regions use alternate pathways — such as C4 and CAM — to capture carbon.
C4 Pathway
Unlike the C3 pathway, the C4 is a bit more complex. As the name suggests, the first stable organic compound produced is a four-carbon compound. This compound, known as oxaloacetate, or malate is formed by the carboxylation of a three-carbon compound, phosphoenolpyruvate (PEP). In this pathway, PEP is the initial CO2 acceptor that receives CO2 in the form of a bicarbonate ion.
The oxaloacetate, the four-carbon organic product, is then transported to bundle sheath cells. Here the compound undergoes decarboxylation and releases CO2, for use in the Calvin cycle — the sugar-making step of photosynthesis.
What About RubP?
You might wonder, “What’s the role of RuBP and Rubisco in the C4 pathway?” Well, not much initially. In the first few stages of the C4 pathway, the primary substrate is PEP, not RuBP. Therefore, without RuBP, there’s no need for Rubisco either. Rubisco cannot catalyze PEP; instead, PEP is catalyzed by an enzyme known as phosphoenolpyruvate carboxylase, or PEPC.
How Does the C4 Pathway Prevent the Loss of Resources?
Apart from the obvious biochemical differences, the saving grace of the pathway lies in the anatomy C4 plants possess—this anatomy is known as the Kranz Anatomy. It’s a bit like Russian Matryoshka nesting dolls. An easy way to visualize the anatomy is to consider mesophyll cells as the outermost layer, followed by bundle sheath cells, and finally vascular bundles. Of these three, the bundle sheath is the most prominent. These cells have thick walls to minimize wasteful processes like photorespiration, conserving both water and CO2!

Features of the Kranz Anatomy
- Division: In C3 plants such as rice, wheat, and oats, carbon fixation and subsequent photosynthetic processes, such as the Calvin Cycle, happen in the mesophyll cells. However, in C4 plants like maize, sugarcane, and certain grasses, processes are separated. Initially, carbon is captured and fixed in the mesophyll cells. It is then transported to bundle sheath cells where the actual Calvin Cycle—the sugar production part—occurs. These bundle sheath cells, specialized structures surrounding vascular bundles containing xylem for water transportation and phloem for nutrient transportation, are adapted to concentrate CO2.
- Efficiency: In C4 plants, carbon fixation occurs effectively twice—first in the mesophyll cells and then in the bundle sheath cells. In the mesophyll, CO2 is fixed into oxaloacetate using the enzyme PEPC. It quickly converts into malate and is transported to the bundle sheath. Here, it undergoes decarboxylation, releasing CO2 (along with pyruvate) once again!
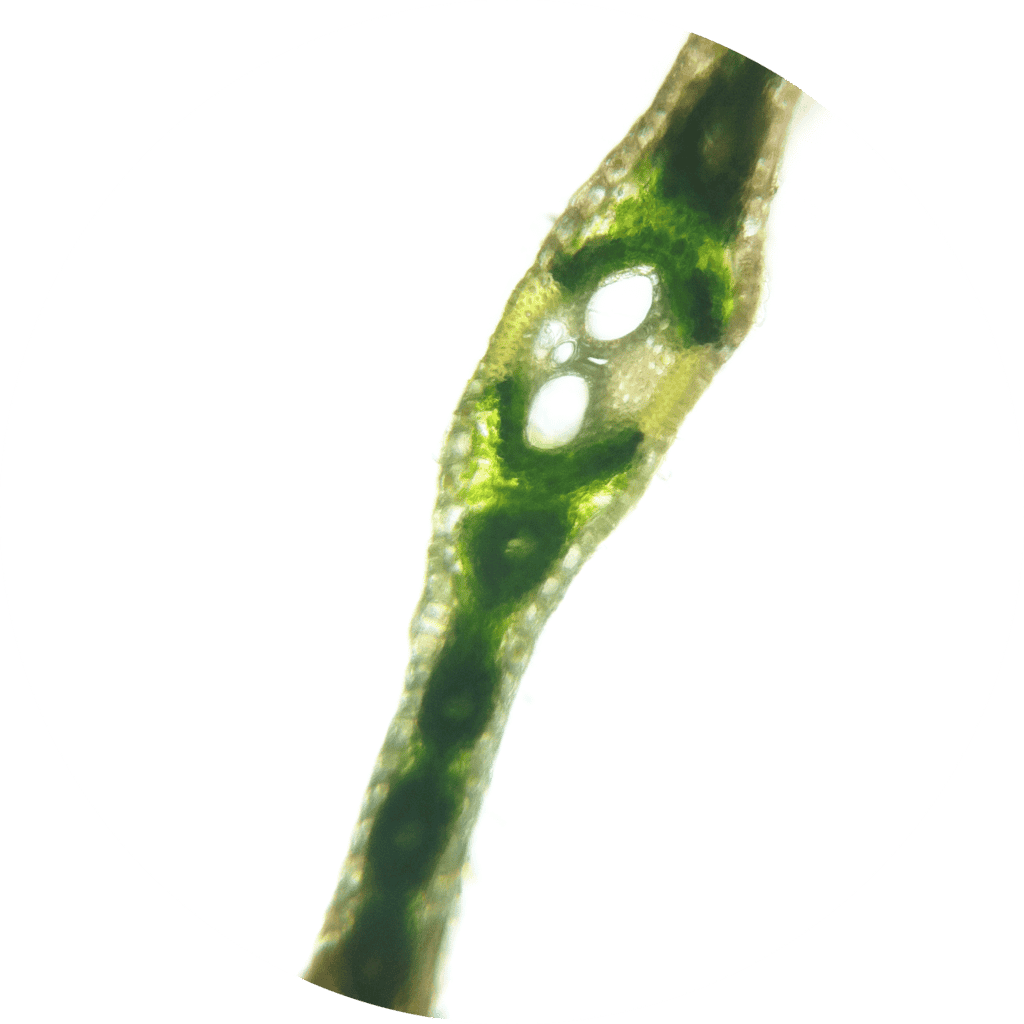
The Second Carboxylation
After malate is metabolized to CO2 and pyruvate, this free CO2 is then used in a second carboxylation. However, instead of using PEP as the substrate, this carboxylation in the chloroplasts of the bundle sheath cells utilizes RuBP. Effectively, this second carboxylation follows a typical C3 pathway. The product of this second carboxylation, 3-PGA, is used for two purposes: glucose (sugar) production as well as the regeneration of RuBP, marking the first and last steps of the Calvin cycle.
CAM Pathway
The Crassulacean Acid Metabolism (CAM) pathway is the last of the fixation pathways. It is a variation of the C4 pathway. Initially discovered in flowering plants of the Crassulaceae family, it is particularly characteristic of succulents and certain aquatic plants. Like the C4 pathway, it conserves water and CO2 by reducing resource loss through photorespiration.

Features of the CAM Pathway
- Storage in Vacuoles: During the night, when stomata are open and atmospheric CO2 is available, CAM plants fix CO2 into organic acids, such as malate, using the PEPC. These organic acids are then stored in the vacuoles of mesophyll cells.
- Nighttime Accumulation: Storing organic acids at night allows CAM plants to avoid water loss through transpiration (water loss through stomata) during the day. This nighttime carbon fixation also reduces the risk of photorespiration.
- C3 Cycle During the Day: During the day, CAM plants use the stored organic acids in the Calvin Cycle (C3 pathway) within the same mesophyll cells. The organic acids undergo decarboxylation, releasing CO2 for use to produce sugars and other organic molecules.
Conclusion
Understanding the intricacies of carbon fixation pathways is challenging yet crucial. This process is one of the remarkable mechanisms within photosynthesis that sustains life on our planet. From the simple C3 pathway to the more intricate C4 and CAM pathways, plants have evolved diverse mechanisms to capture and utilize atmospheric carbon dioxide.
In essence, carbon fixation is indispensable for synthesizing organic molecules, serving as fundamental building blocks for plant growth and metabolism. It plays a pivotal role in the global carbon cycle, supporting ecosystems and life on Earth.